INTRODUCTION
Working memory (WM), the ability to actively store useful information “in mind” over seconds, plays a vital role in many cognitive functions. Individual differences in WM capacity predict fluid intelligence and broad cognitive function (1), which has made increasing WM capacity become an attractive aim for interventions
and enhancement. In the past decades, noninvasive brain stimulation (NIBS) technology involving transcranial application of electrical (direct or alternating) or magnetic fields to the specific scalp or multiple brain circuits has been proven to be useful for improving WM performance. Research using NIBS has found that behavioral
enhancement is associated with neurophysiological changes, including increased interregional functional connectivity (2) and oscillatory neuronal activity (3) as well as changes in event-related potentials (ERPs) (4). Transcranial photobiomodulation (tPBM) is a noninvasive light illumination method that targets the brain at wavelengths between 600 and 1100 nm. Recently, tPBM has been applied to modulate metabolic processes in the brain, and it has emerged as a promising intervention to improve cognitive functions. It has been suggested that tPBM up-regulates complex IV of the mitochondrial respiratory chain to modulate cytochrome c oxidase (CCO). This leads to increased adenosine triphosphate (ATP) formation and initiates secondary cell signaling pathways (5–7). The resulting metabolic effects following PBM increase cerebral metabolic energy production, oxygen consumption, and blood flow in animals and humans (8–11). In addition, several studies suggest that PBM can (i) enhance neuroprotection by modulating neurotrophic factors and inflammatory signaling molecules as well as anti-apoptotic mediators (12), (ii) activate ion channels (13), and (iii) stimulate transcription factors that up-regulate the expression levels of genes (14). In an Alzheimer’s disease (AD) mouse model, tPBM can reduce perivascular microglia and promote angiogenesis to further enhance
A clearance (15). In particular, in the past 2 years, several clinical studies have provided convincing evidence that tPBM improves cognition in patients with AD and dementia (16, 17) and facilitates treatment for other neurological disorders (18, 19). Regarding WM, Rojas et al. (20) demonstrated that tPBM could improve prefrontal cortex (PFC) oxygen consumption and metabolic energy, thereby increasing PFC-based memory functions in rats. Other studies have shown that 1072-nm tPBM can reverse WM deficits in middle-aged mice (21). These animal findings suggest that the oxygen metabolism of cortical tissue exposed to tPBM is enhanced and that this can explain the improved memory. Two human behavioral studies have shown that 1064-nm tPBM over the right PFC can improve accuracy and speed up reaction time in WM tasks (22, 23). Meanwhile, other behavioral studies suggested that high-order cognitive functions, such as sustained attention and emotion (22), as well as executive functions (24), could also be improved after tPBM therapy.
However, even the simplest WM task involves multiple cognitive processes, such as perceptual encoding, selective attention, and motor execution, which might confound the associations between the tPBM effect and WM enhancement. Taking this into account, we chose the K values estimates to assess the accurate number of items maintained in the visual WM for the given load array (25). Given that the right PFC is associated with information maintenance in WM (26), we hypothesized that 1064-nm tPBM over the right PFC (Fig. 1A) leads to enhancements in visual WM capacity. However, we still lack WM-related neurological evidence to directly
bridge the gap between tPBM effects and WM behavioral benefits. Previous studies have extensively demonstrated that contralateral delay activity (CDA) tracks the number of objects stored in visual WM. Furthermore, the set-size effects of the CDA (defined as the increase in amplitudes from set-size two to set-size four) predicted the individual differences in WM capacity (27). Thus, we linked behavioral benefits (K values) in WM capacity from tPBM with ERP biomarkers (CDA) of WM capacity. As the research on tPBM as a
potential tool for effective NIBS is in its early stages, several key questions exist. For example, a good understanding of tPBM-evoked electrophysiological effects in the human brain is lacking.
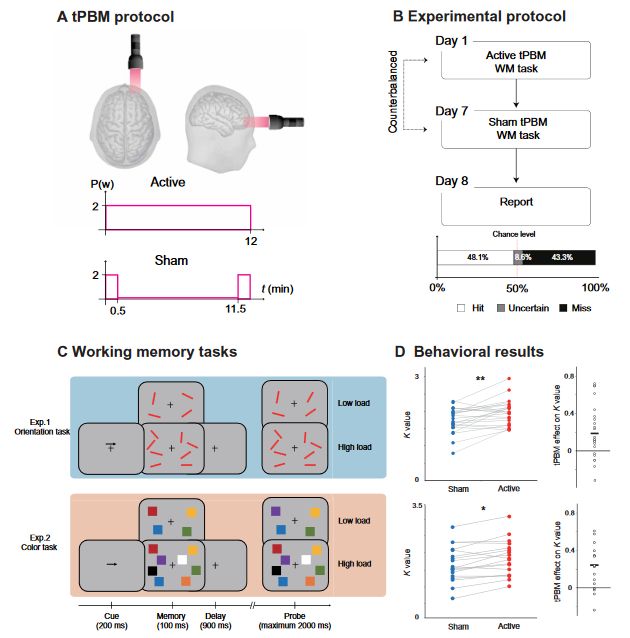
Fig. 1. Protocol, task, and behavioral results in experiments 1 and 2. (A) tPBM protocol. Active tPBM was delivered by a laser with 1064 nm to the right PFC for a total of 12 min. (B) Experimental protocol. Each participant received two tPBM sessions (active and sham, randomized, and double-blinded design) separated by 1 week. On the eighth day, participants were required to report or guess which session involved active or sham tPBM. (C) WM tasks. In experiment 1, the participants were required to perform an orientation WM task. In experiment 2, the participant was required to perform a color WM task. Two tasks used the same relative timing and protocol, and the only difference between the two tasks was the memory dimension (orientation in experiment 1 and color in experiment 2). Each participant only participated in one experiment. (D) Left: Performance in terms of K values for orientation WM task (up) and color WM task (down) under sham tPBM (blue circles) and active tPBM (red circles). Right: The tPBM effect on the K values (active minus sham). The dots indicate individual performance. *P < 0.05 and **P < 0.01.
We conducted four double-blind, sham-controlled tPBM experiments (Fig. 1A), in which participants completed two different sessions of tPBM separated by a week, with sham or active tPBM on the PFC (Fig. 1B). After stimulation, participants performed a classical change detection task in which WM load was manipulated (high versus low load; Fig. 1C), while the electroencephalography (EEG) was recorded. The classical change detection task required participants to maintain the features of items (orientation for experiment 1; color for experiment 2) at the cued side in WM for subsequent recognition, which reliably induces sustained CDA components. Then, we performed a series of follow-up experiments that explored the specificity of tPBM in terms of wavelengths (experiment 3) and stimulation site (experiment 4) on the enhancement of WM capacity.
RESULTS
1064-nm tPBM on the right PFC enhanced individual WM capacity
Two classic change detection tasks were implemented to assess WM performance, which required the participants to remember the orientations (experiment 1) or color (experiment 2) of a set of items in
the cued hemifield (see Fig. 1C). We calculated the visual memory capacity using a standard formula (27) that essentially assumes that if an observer can hold in memory K items from an array of S items, then the item that changed should be one of the items being held in memory on K/S trials, leading to correct performance on K/S of the trials in which an item changed. The formula for memory capacity is K = S × (H − F), where S is the size of the presented array, H is the observed hit rate, and F is the false alarm rate. We evaluated the WM capacity according to the K values under the high-load condition. A two-way mixed-effect analysis of variance (ANOVA) with tPBM stimulation (active and sham; within-subjects) and tasks (orientation and color; between-subjects) as factors was conducted with K values as the dependent variable. The results revealed a significant main effect of tPBM stimulation (F1,40 = 13.436, P < 0.001, P2 = 0.925) but no significant interaction between tPBM stimulation and task (F1,40 = 0.080, P = 0.779, P2 = 0.006). Specifically, compared with sham tPBM, the K values increased after 1064-nm tPBM both in the orientation WM task (experiment 1: t22 = 2.841 and P = 0.009, two-tailed) and in the color WM task (experiment 2: t17 = 2.760 and P = 0.013, two tailed). The mean tPBM effect (active minus sham) on the K values for experiment 1 was 0.186 ± 0.065
(BF10 = 5.212 and Cohen’s d = 0.568), and the mean tPBM effect on K values for experiment 2 was 0.188 ± 0.051 (BF10 = 20.336 and Cohen’s d = 0.651). These results support the hypothesis that 1064-nm tPBM on the right PFC enhances WM capacity. Several studies investigating neuro-enhancement using transcranial direct current stimulation (tDCS) have shown improved WM with stimulation but mainly for individuals with low WM capacity (4, 28). To examine this effect with respect to tPBM, we divided participants into two subgroups based on their K values in the orientation WM task during sham tPBM stimulation in experiment 1
(n = 11 and n = 12 for low- and high-performance groups, respectively). A two-way mixed-effects ANOVA showed no significant interaction between tPBM stimulation and subgroup (F1,22 = 1.170, P = 0.291, P2 = 0.110). Therefore, participants with both good and poor WM capacity improved after 1064-nm tPBM. A similar analysis involving the color WM task also showed no performance-dependent effects in experiment 2 (F1,17 = 0.002, P = 0.963, P2 < 0.001). Our results in experiments 1 and 2 demonstrate that participants could maintain more items in visual WM with external 1064-nm tPBM stimulation on the right PFC. These effects were independent of prior WM ability for both orientation and color. Participants could not report
or guess whether they were assigned to the sham or active tPBM group. Subjects guessed at chance level (see Fig. 1B, hit rate = 48.1%), suggesting that they had no awareness of whether they received active tPBM.
CDA tracks the enhancement in individual WM capacity
The EEG signals were recorded while participants performed the WM tasks. Consistent with previous studies (27), the ERP results show a negative deflection at contralateral relative to ipsilateral scalp sites at PO7 and PO8 (see the Supplementary Materials). We defined the CDA set-size effect as the CDA amplitude with respect to the maintenance of S = 2 objects (low load) minus the amplitude of maintenance of S = 4 objects (high load). Note that we did not track the “raw” CDA amplitude but rather used the increase in CDA amplitude from low to high WM load as the dependent vari- able. To investigate the effect of tPBM on the CDA set-size effect, a two-way mixed ANOVA on the CDA set-size effect was conducted considering the WM task (orientation and color) and tPBM stimulation (active and sham) as factors. As expected, the results showed a significant main effect of tPBM stimulation (F1,40 = 12.249, P = 0.001, P2 = 0.227). The main effect of task (F1,40 = 0.660, P = 0.421, P2 = 0.012) and the tPBM stimulation × task interaction (F1,40 = 0.474, P = 0.495, P2 = 0.011) was not significant. Follow-up t tests indicated that the CDA set-size effect during the delay period was significantly stronger in the active tPBM session than in the sham tPBM session for both the orientation WM task (t22 = 2.313, P = 0.030, Cohen’s d = 0.463, two-tailed) and color WM task
(t17 = 2.506, P = 0.023, Cohen’s d = 0.591, two-tailed). Source estimates using standardized low resolution electromagnetic tomography (sLORETA; see Materials and Methods) of the CDA set-size effect are shown in Fig. 2. These results demonstrated that the increase in CDA set-size effects (active minus sham) was localized in the superior intraparietal sulcus (IPS) for two WM tasks with 1064-nm tPBM applied over the right PFC (Ps < 0.05). To better understand the tPBM effect, we show the K values and CDA set-size effects for the different stimulation sessions with respect to the orientation and color WM tasks (Fig. 2C). Although the K values and CDA set-size effects were evaluated as separate dimensions involving WM, as can be seen here, the changes in K values and CDA across the two tasks have similar trends as when compared active tPBM to the sham tPBM. These results are consistent with the hypothesis that both WM improvement and CDA increases are associated with the tPBM effect. As expected, the CDA set-size effect from the two experiments shows a task-independent tPBM effect. Next, we tested whether the EEG data provided evidence of the beneficial tPBM effect. We performed Pearson correlation analyses at the subject level to provide more detailed information on the relationships between CDA and behavior. As shown in Fig. 2D, the differences in CDA set-size effects between active and sham sessions were correlated positively with the behavioral differences between these sessions (for orientation task: r = 0.446 and P < 0.040; for color task: r = 0.563 and P < 0.020). The results suggest that, for both color and orientation WM tasks, the tPBM-associated changes in CDA set-size effects can predict tPBM-associated behavioral benefits.
CDA mediates the WM improvement with tPBM (1064 nm) applied to the right PFC
Given the above notable relationship between the increase in CDA set-size effect and the increase in K values after 1064-nm tPBM, relative to sham sessions, we performed a mediation analysis (see Materials and Methods) to examine whether the effect of tPBM on WM capacity (reflected by K values) was mediated by the CDA set-size effect. Therefore, we considered the tPBM sessions (active versus sham) as predictors, WM performance (K values) as the predicted variable, and CDA set-size effect as a mediator (see Fig. 3A). This mediation analysis revealed a significant indirect effect of the CDA set-size effect (indirect effect: 0.300, 95% confidence interval: −0.107 to 0.706, P = 0.146; direct effect: 0.245, 95% confidence interval: 0.021 to 0.844, P = 0.040). The mediation analysis demonstrated the indirect effect of 1064-nm tPBM on the behavioral K values through an increase in the amount of information maintained in visual WM, as reflected by increases in the CDA set-size effect. We further correlated the CDA set-size effect with the K values across all sessions in experiment 1 and experiment 2. The correlation analysis showed that the change in the CDA set-size effect was strongly correlated with the change in the behavioral K values (r = 0.404, P < 0.001, confidence interval: 0.154 to 0.654; Fig. 3B), highlighting a robust relationship between the CDA set-size effect and WM improvement. This result is consistent with previous research (29) that CDA is indicative of the number of maintained objects in visual WM.
The wavelength specificity of tPBM on the enhancement of WM capacity
In experiment 3, we investigated whether the changes in neural activity and behavior were specific to the wavelength of tPBM.
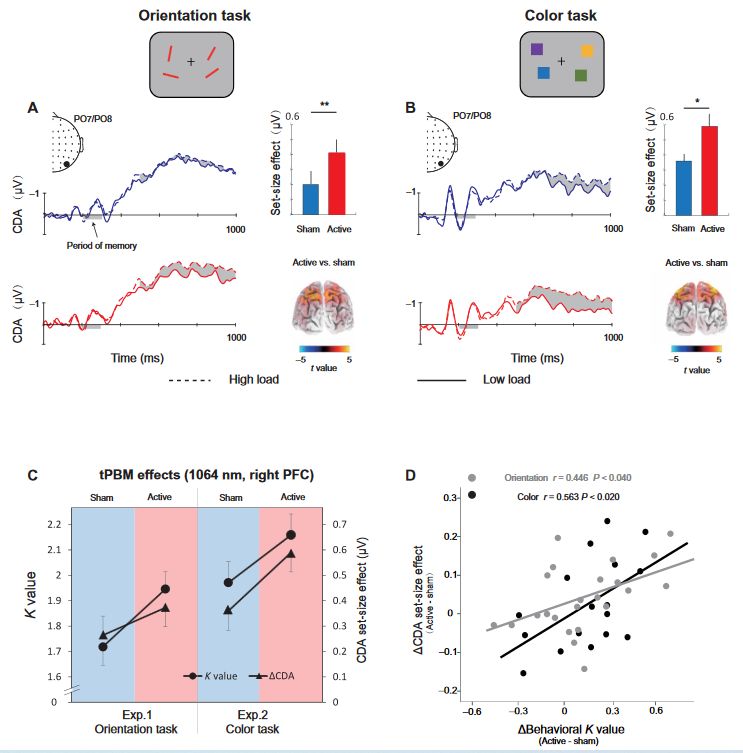
Fig. 2. Grand average of ERPs and its link with behavior. (A) The orientation WM task in experiment 1 and (B) the color WM task in experiment 2. Shading indicates the CDA set-size effect. The enlarged black dots on EEG topographies show PO7/PO8 electrodes. Bar plots represent the average CDA set-size effect. Error bars represent SEM. Significant set-size effects are located in the IPS. Three-dimensional (3D) brain map (t-map; posterior view) of significant tPBM effect on CDA. (C) tPBM-effect. K values and CDA set-size effect for the two tasks (orientation WM task and color WM task) and two sessions (active tPBM and sham tPBM). (D) Scatterplots of participants’ behavioral benefits (active minus sham) against the changes in the CDA set-size effect (active minus sham) for the orientation WM task (gray) and the color WM task (black). *P < 0.05 and **P < 0.01.
approximate wavelength of 850 nm has been used in near-infrared light for testing WM improvements due to its absorption maxima of CCO (9). This motivated us to test tPBM by using 852-nm laser light over the right PFC (Fig. 4A). We also sought to disambiguate the effects of tPBM and tissue heating. We hypothesized that if tPBM on the enhancement of WM capacity is not wavelength specific, but temperature dependent, then we should expect to find about the same enhancement in K values and CDA set-size effect with 852-nm tPBM and 1064-nm tPBM. We used the same power and stimulation duration for 1064-nm tPBM and 852-nm tPBM to ensure that they produced the same quantity of heat. First, we found that 852-nm tPBM did not change the participants’ behavioral K values for the orientation WM task compared with sham tPBM (t18 = 0. 381, P = 0.707, Cohen’s d = 0.085, two-tailed; Fig. 4B). The mean tPBM effect (active minus sham) on the K values for experiment 3 was −0.029 ± 0.088 (BF10 = 0.244 and Cohen’s d = 0.085). We compared the data between the 852-nm tPBM in experiment 3 and the 1064-nm tPBM in experiment 1 (Fig. 4D). A two-way
mixed-effect ANOVA on K values further revealed a significant tPBM stimulation (active versus sham) and wavelength (1064 nm versus 852 nm) interaction (F1,41 = 4.474, P = 0.041, P2 = 0.095), indicating that WM performance improved significantly only in
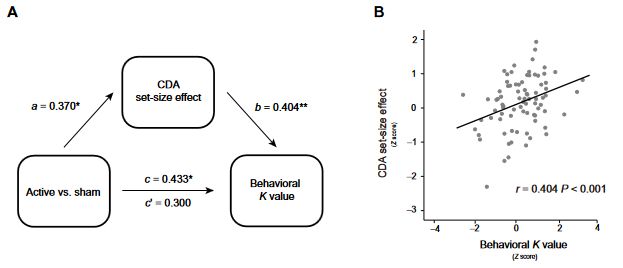
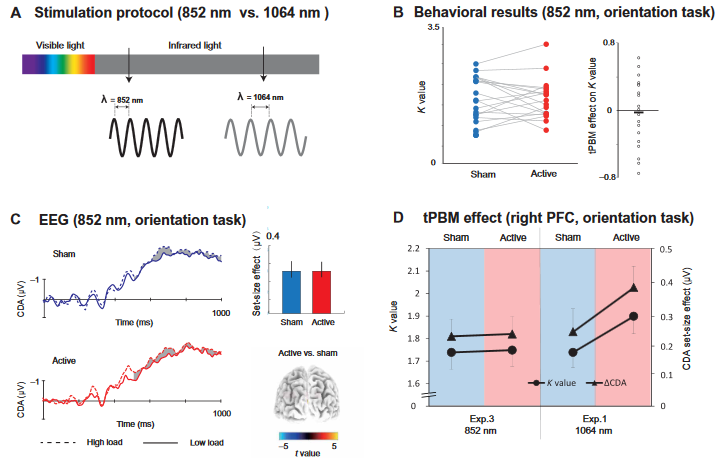
active tPBM sessions applying 1064 nm but not an 852 nm. Similarly, 852-nm tPBM did not modulate the CDA set-size compared with sham tPBM for the orientation WM task (t18 = 0. 129, P = 0.899, Cohen’s d = 0.030, two-tailed; Fig. 4C). A two-way mixed-model ANOVA on CDA amplitudes further revealed a marginally significant tPBM stimulation (active versus sham) and wavelength (1064 nm versus 852 nm) interaction (F1,41 = 3.623, P = 0.064, P2 = 0.080). These results suggest that the tPBM effect on WM is specific to the 1064-nm wavelength. We conclude that the behavioral and electrophysiological findings with 1064-nm tPBM are not explained by heating. This conclusion should be interpreted with caution due to the marginal significance observed in our electrophysiological results. Subjects also guessed at the chance level (hit rate = 47.8%), suggesting that they had no awareness of the 852-nm tPBM over the right PFC.
1064-nm tPBM on the left PFC did not enhance WM capacity
In experiment 4, we asked whether 1064-nm tPBM applied to the left PFC could enhance WM capacity (Fig. 5A). The task in experiment 4 is the same orientation WM task as experiment 1. Figure 4 shows that compared with sham tPBM, 1064-nm tPBM on the left PFC did not enhance the K values (t18 = 0. 381, P = 0.707, Cohen’s d = 0.085, two-tailed; Fig. 5B) nor the corresponding CDA set-size effect (t18 = 0. 129, P = 0.899, Cohen’s d = 0.030, two-tailed; Fig. 5C). The mean tPBM effect (active minus sham) on the K values for experiment 4 was −0.032 ± 0.058 (BF10 = 0.258 and Cohen’s d = 0.030). We compared the data between tPBM applied on the left PFC in experiment 4 and tPBM applied on the right PFC in experiment 1
(Fig. 5D). A two-way mixed-model ANOVA revealed significant interactions between tPBM stimulation (active versus sham) and location (left versus right) on both K values (F1,41 = 4.474, P = 0.041, P2 = 0.095) and on the CDA set-size effect (F1,41 = 2.623, P = 0.044, P2 = 0.098). These findings demonstrate that WM performance improved only when 1064-nm tPBM was applied to the right but not to the left PFC. Subjects also guessed at the chance level (hit rate = 46.9%) whether they received sham versus tPBM, suggesting
that they had no awareness of the tPBM over the left PFC.
Time course of the enhancement in WM after tPBM
To investigate the behavioral enhancement across blocks, we calculated the K values of high-load conditions across the four blocks in all four experiments (Fig. 6). The paired t test showed that, relative to sham sessions, significant behavioral enhancements were found
only during the late period in experiment 1 (block 3: t 22 = 3.840,
P < 0.001, Cohen’s d = 0.768, two-tailed; block 4: t22 = 2.155, P = 0.041, Cohen’s d = 0.504, two-tailed) and experiment 2 (block 3: t17 = 2.137, P = 0.047, Cohen’s d = 0.431, two-tailed) with 1064-nm tPBM on the right PFC but not in other blocks or experiments (Ps > 0.250). We further compared the K values in block 3 across experiment 1 and experiment 3 when applying tPBM over the right PFC, 1064-nm stimulation relative to 852 nm enhanced the K values (t40 = 2.795, P = 0.008, Cohen’s d = 0.838, two-tailed). When applying tPBM at 1064-nm wavelength over the right PFC relative to the left PFC, the K values were also enhanced (t40 = 1.959, P = 0.056, Cohen’s d = 0.580, two-tailed). No significant differences were found in other blocks (Ps > 0.351).
No evidence for event-related oscillatory activity after tPBM
We further analyzed the effects of tPBM on event-related desynchronization (ERD) or event-related synchronization (ERS) during the retention of WM. As shown in fig. S1, we found that active
tPBM might amplify the set-size effect of theta ERS in experiment 1 (t22 = 2.487, P = 0.048, Cohen’s d = 0.403, two-tailed). This effect was not found in experiments 2, 3, and 4 (Ps > 0.256). We further
performed a correlation analysis to determine whether the set-size effect of prefrontal theta power was correlated with behavioral benefits on WM capacity in experiment 1. No significant correlation was found between theta ERS and changed in K values (r = 0.232 and P = 0.351). As shown in figs. S2 and S3, no significant differences in the set-size effect of alpha or beta ERD between the two tPBM
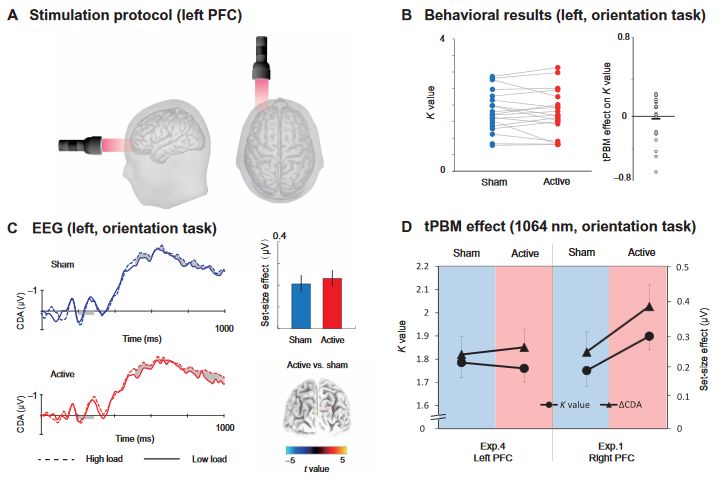
Fig. 5. Results in experiment 4. (A) Stimulation protocol of experiment 4. Active tPBM was delivered by the laser with wavelength 1064 nm at the left PFC for a total of 12 min. (B) K values for tPBM stimulation (active and sham) were applied on the left PFC in experiment 4. Each circle indicates individual performance. (C) Grand average of ERPs for the 1064-nm and sham tPBM sessions in experiment 4. Shading indicates the CDA set-size effect. Bar plots represent the average CDA set-size effect: blue, sham session; red, active session. Error bars represent SEM, 3D brain map (t-map) posterior view of a significant tPBM effect on CDA. (D) K values and CDA set-size effect for the orientation WM task in experiment 4 (tPBM stimulation applied on the left PFC) and in experiment 1 (tPBM stimulation applied on the right PFC).
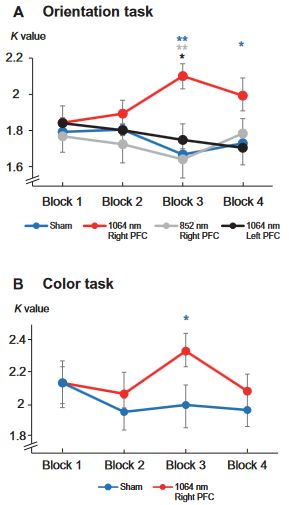
sessions were found in each experiment (Ps > 0.188). No significant relationships were found between the changes in the set-size effect of alpha (or beta) ERD and behavioral benefits (Ps > 0.150). The ERD or ERS modulations were also not linked to the changes in the CDA set-size effect with stimulation. The reason might be as follows: (i) although both oscillatory activities and CDA are related to WM capacity (27, 30), some studies further identified that they reflect dissociable processes of WM (31, 32); (ii) poor statistical power for the oscillatory analysis explained by a lower signal-to noise ratio. Recent findings proposed that cross frequency coupling (e.g., phase-amplitude coupling) and long-range connections between distant cortical areas (e.g., phase locking value) might be related to network mechanisms supporting WM maintenance (2, 3).
Further work is needed to examine the tPBM effect on network changes during WM.
DISCUSSION
Across four complementary EEG experiments, we provided the converging evidence that 1064-nm tPBM applied to the right PFC could improve visual WM capacity. In the first two experiments (experiments 1 and 2), the K values were enhanced for WM for orientation and color when 1064-nm tPBM was applied to the right PFC. Crucially, we found that the WM memory improvements were tracked by individual changes in CDA set-size effects. A mediation analysis revealed that the behavioral enhancements with tPBM were mediated by CDA set-size effects. Further studies demonstrated that effects on the capacity enhancement of visual WM were absent for tPBM applied at 852-nm tPBM (experiment 3) and to the left PFC (experiment 4)
Improvement of CDA-identified WM capacity by 1064-nm tPBM on the right PFC
There has been recent excitement about tPBM as a safe, noninvasive, and simple modality for neuromodulation. In particular, it seems to be a promising tool for enhancing WM capacity in humans because several studies have shown notable improvements in tPBM-treated versus control groups as measured by behavioral responses in WM tasks (22, 23). Our results from experiments 1 and 2 do not only
complement those findings, but also the design and outcome of these two experiments add novel insight.
First, WM involves multiple cognitive processes, including perceptual encoding, selective attention, and motor execution. More specific measures of WM capacity (e.g., K values and CDA set-size
effect) bring us closer to identifying specific enhancements by tPBM. Thus, we designed an EEG study to investigate the post-tPBM effects on the CDA reflecting the individual visual WM capacity. Because the CDA set-size effect reflects the number of objectsonline-held in visual WM (29), our ERP results help to establish
that 1064-nm tPBM on the right PFC boosts visual WM capacity. It also corroborates and extends existing findings that active maintenance of visual information in the occipitoparietal cortex could be boosted by enhancing the contribution of the right PFC in visual WM maintenance (33). We further established neurophysiological links between 1064-nm tPBM and subsequent WM capacity, by demonstrating that the CDA during the retention served as a mediator. Our findings suggest that increased WM from 1064-nm tPBM might stem from the right PFC stronger engaging parietal areas as reflected by the increased CDA set-size effect. However, given that both hemodynamic effects (34) and EEG activity (35) from the IPS
are correlated with WM capacity, we could not pinpoint whether the CDA set-size effect plays a causal role in enhancing WM capacity or whether it is a byproduct of hemodynamic activity. Given that the frontoparietal network (FPN), including the supplementary motor area, PFC, and IPS are thought to be important for WM (36), we inferred that the 1064-nm tPBM might increase metabolism (e.g., providing more ATP) in the right PFC with positive benefits for the FPN network. Second, EEG signals in response to tPBM stimulation have been reported recently from resting brain data, and they were analyzed to identify frequency-specific changes in EEG power (16, 37, 38). Our study demonstrates that WM-specific ERPs also are modulated by tPBM stimulation. This contribution is important for researchers working in the field of tPBM who wish to understand the underlying electrophysiological effects of tPBM.
Possible changes in the brain network by 1064-nm tPBM in the right PFC
The LORETA results showed an effect of ERPs from the IPS when the stimulation was applied over the right PFC. There are several explanations for this observation. A recent study (38) reported that 1064-nm tPBM on the right PFC enables notable increases in alpha power in several brain networks at rest (i.e., the default mode
network, executive control network, frontal-parietal network, and lateral visual network). Another independent set of studies from the same group revealed that 1064-nm tPBM on the right PFC increased
hemodynamic activities across the entire cortical region and en- hanced topographical functional connectivity between the right PFC stimulation site and parietal regions (5, 39). Together, these studies show that 1064-nm tPBM on the right PFC of the resting human brain modulates both regional-specific activity and functional connectivity as observed in EEG and hemodynamic data. While these results were derived from the intervention administered during the resting state, it is reasonable to expect that tPBM would also alter
the task-related activity between the right PFC and the parietal regions as we here report. We posit that the CDA set-size effect that we report is related to connectivity changes between the right PFC stimulated by
tPBM and the IPS generating the CDA. In support, it has been suggested that external interventions can result in increased functional connectivity between the PFC and IPS during WM operations (3, 40).
The tPBM applied in this study is likely to result in changes and enhancement in metabolism and hemodynamics (41, 42). It has been suggested that the neurovascular coupling between hemodynamic activity and EEG is suggested to play a role in visual informationprocessing (43, 44). Thus, further EEG functional near-infrared spectroscopy (fNIRS)/functional magnetic resonance imaging (fMRI) studies would help to gain a better understanding of the underlying mechanism of the beneficial effects resulting from tPBM.
Read full article here: https://www.science.org/doi/pdf/10.1126/sciadv.abq3211